GENERAL SUMMARY OF OUR RESEARCH
Our lab aims to understand—at the molecular, cellular, and organismal levels—how protein unfolding in the endoplasmic reticulum (ER) causes human disease, in order to develop new therapeutic approaches for these conditions.

DETAILED SUMMARY OF OUR RESEARCH
An ER intracellular signaling pathway called the unfolded protein response (UPR) ensures that the machinery needed to fold and assemble secreted and membrane proteins is sufficient to meet cellular secretory needs. When the ER's protein folding needs outweigh its capacity to sustain the protein folding process, cells experience a distinct form of stress—"ER stress"— that triggers the UPR pathway. UPR outputs initially reduce ER stress through augmenting the levels of ER-resident chaperones and protein modification enzymatic activities; these outputs are conserved in all eukaryotes. However, if ER stress cannot be alleviated through these adaptive outputs, in higher eukaryotes such as mammals, the UPR triggers a diametrically opposite strategy to instead promote destructive outcomes, including sterile inflammation, dedifferentiation, and ultimately programmed cell death (Molecular Cell, 69(2), 2018).
Over the last 15 years, our lab was the first to elucidate how such a pivotal ER stress-induced life-death switch is controlled in mammalian cells by the master UPR sensor/effector, IRE1alpha, an ER trans-membrane bifunctional kinase/RNAse protein. While several other labs have defined the adaptive benefits proceeding through IRE1alpha, our lab was the first to show that IRE1alpha has alternative outputs—both adaptive and destructive—depending on the strength of upstream stresses; these “Janus-faced” distinctions of IRE1 function are key to shaping therapy. For instance, in 2009, we were the first to find that IRE1alpha has alternate RNAse outputs that are controlled by its kinase domain to promote divergent cell fates under ER stress (Cell, 138, 562–575, 2009). In that study we showed that hyperactivated IRE1alpha caused the ER-localized degradation of myriad mRNAs—including those encoding ER chaperones—in mammalian cells and the consequent depletion of these mRNAs leads mammalian cells to enter apoptosis. Then in 2012 we identified a critical node in the UPR called the thioredoxin-interacting protein (TXNIP), which promotes sterile inflammation in pancreatic islet beta cells to cause development of diabetes (Cell Metabolism, 16(2): 250-64 (2012). Furthermore, we learned that TXNIP is induced post-transcriptionally by IRE1alpha as IRE1alpha degrades a set of pro-oxidant and apoptosis-inhibitory micro RNAs, such as miR-17 (Science 338 (6108) (2012)). Such post-transcriptional control may be widespread in the UPR. The recurring theme in our studies is that a transition from an adaptive to a terminal UPR promotes destructive outcomes in cells experiencing irremediably high and chronic levels of ER stress.
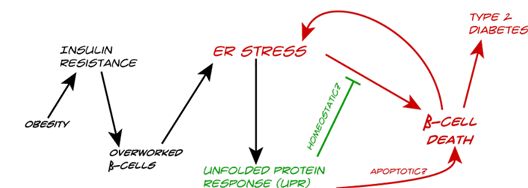
The reason we are so interested in these topics is that ER stress-mediated apoptosis is being widely linked to many important human diseases that currently have no cure. Overall, these ER stress-mediated diseases occur when specialized cells in the body that evolved to secrete proteins—"professional secretory cells"— die prematurely. We started our studies focusing particularly on diabetes mellitus as a prototype of ER stress-mediated cell degenerative diseases, but have branched our work to many other conditions—(see below). For example, the pandemic disease type 2 diabetes is known to occur when about 50% of pancreatic islet beta cells (specialized cells in the pancreas that synthesize and secrete insulin) die, leading to insufficient insulin levels in the blood. We hypothesize that pancreatic islet beta cells may become overworked as they try to counter peripheral insulin resistance in states of obesity and overnutrition (high fat and high carbohydrate diets). Overwork of these cells may cause them to experience elevated ER stress chronically. In turn, elevated ER stress puts the beta cells at heightened risk for death as the UPR starts triggering apoptosis. As more beta cells die, the remaining beta cells experience greater and greater ER stress because they have to do more work per cell. Similar logic may apply in type 1 diabetes, as beta cells are subject to autoimmune attack. This chain of events leads to a vicious cycle of beta cell loss as diabetes sets in. However, the process of disease progression may take many years, providing us with a long time-window to reduce, and possibly even to reverse, the course of the disease.
Ultimately, our long-term goals are to advance fundamental knowledge in order to develop new drugs to reduce maladaptive and destructive responses (e.g. premature apoptosis) occurring in ER stressed cells. It is conceivable that such drugs may be efficacious in diabetes, but may also be useful for many other ER stress-mediated cell degenerative diseases. The basic questions we ask include: is there a "tipping point" of ER stress beyond which the UPR relegates beta cells to apoptosis? And can we intervene through pharmacology to adjust that tipping point, and reduce cell death?
To these ends, we have learned to actively manipulate the UPR pathway in living cells, via cell-permeable small molecules. Using chemical-genetics, we first employed “bump-hole” small molecule tools to wrest pharmacological control over the UPR in baker’s yeast—in the process we discovered an unprecedented paradigm of kinase signaling wherein engagement of an allele-sensitized IRE1 with a bumped kinase inhibitor called 1NM-PP1 unexpectedly bypasses phosphotransfer activity to directly activate the RNAse catalytic activity. (Science 302, 1533-1537 (2003)). Thus, built into IRE1 proteins we learned that there is a conformational switch that controls the RNAse through a pseudokinase module. Next, we discovered that similar engagement of mammalian IRE1alpha confers cytoprotection in mammalian cells experiencing irremediable ER stress (BBRC 365 (2008—published online in 2007) 777–783).
Furthermore, we devised biosensors for measuring ER stress, independently of the UPR, in single living cells, in real time, through employing redox-responsive fluorescent protein sensors—called eroGFP—as a proxy for the ER stressed state (Cell, 135(5):933-47 (2008)). Using eroGFP, we first discovered an ER stress-mediated chaperone-mediated pathway that removes folded ER-resident proteins back to the cytosol—we named this novel process “ER Reflux” (P.N.A.S., 116(23), 2019). While we first discovered ER Reflux in baker’s yeast, the ER Reflux pathway may have evolved to be wired into the life-death decisions that mammalian cells make under ER stress.
Finally, having identified IRE1alpha’s RNase as a life-death switch, we discovered—and are continually optimizing—small molecules that extract desired outputs from this effector domain. As IRE1alpha’s RNase can be controlled from a distance (allosterically) through the kinase domain, we learned in 2012 how to both turn ON, or turn OFF, IRE1alpha at will: we discovered that certain small molecule kinase inhibitors (KIs) of IRE1alpha can elevate RNase activity (called type I) without ER stress, or attenuate it (called type II) even during ER stress—(Nature Chemical Biology, 12: 982-9 (2012)). The distinction between these two classes of compounds is that type 1 KIs actively promote IRE1alpha homo-oligomerization, while type II KIs break homo-oligomerization.
We expect that these two classes of IRE1alpha-modulatory small molecules will allow us to tilt the cell fate outcome in ER stressed cells in opposite directions: either towards survival or death, and therefore these kinase inhibitory compounds will be powerful tools to investigate connections between ER stress, the UPR, cell survival and apoptosis. Importantly, we are the first to develop and employ the type II class of kinase inhibitors of IRE1alpha (which we named KIRAs for Kinase-Inhibiting RNAse Attenuators) as a general proof-of-concept for treating ER stress-related diseases in animal models of ER stress-induced disease. In 2014 we showed that in rodent models of ER stress-induced retinal degeneration and diabetes, a first-in-class compound series of KIRAs ameliorates cell death and preserves physiological function (Cell 158: 534-48 (2014)). This was the first in vivo pharmacological demonstration that hyperactivated IRE1alpha drives myriad cell degenerative diseases (and which can be modified through small molecule inhibitors of IRE1alpha) and represents a departure from a more entrenched view that IRE1alpha outputs are universally beneficial. Since then we have shown that highly-potent and selective KIRAs can reverse autoimmune (type 1) diabetes (Cell Metabolism, 2017, 25(4)) and pulmonary fibrosis (PLoS One, 2019, 14(1)), in pre-clinical mouse models of these diseases.
Encouraged by these results, we are currently advancing KIRAs through medicinal chemistry efforts to make them more drug-like for many other animal and pre-clinical studies of ER stress-related diseases, including diabetes, retinal degeneration, and the currently incurable disease, idiopathic pulmonary fibrosis. Also, several important neurodegenerative diseases, such as Alzheimer's disease, Parkinson's disease, and Lou Gehrig's disease, result from protein unfolding and aggregation in different types of neurons. Hallmarks of high ER stress are apparent in the affected neurons. It is therefore conceivable that small molecule drugs that reduce ER stress and terminal UPR signaling such as KIRAs may show benefit in pre-clinical models of these pathologies and could eventually be advanced to the clinic to test their therapeutic efficacy in human patients suffering from these deadly neurodegenerative diseases.